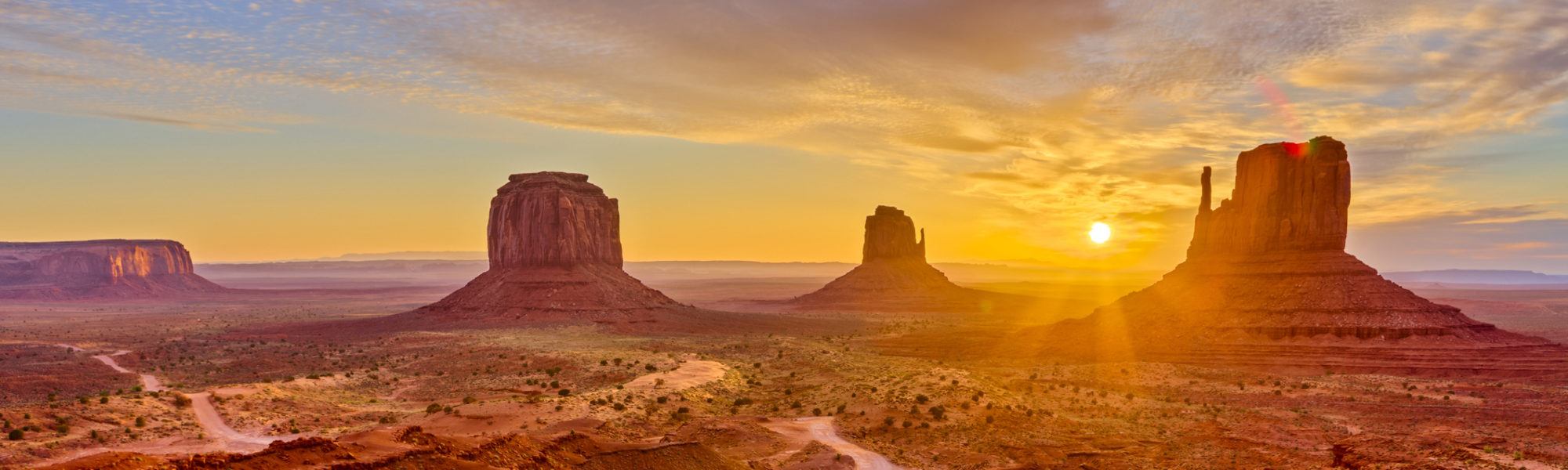
Current Clinical Applications of MR Spectroscopy of the Brain
Authors
Bradley J. Bohnert, MD
John P. Karis, MD
Division of Neuroradiology, Barrow Neurological Institute, St. Joseph’s Hospital and Medical Center, Phoenix, Arizona
Abstract
Magnetic resonance (MR) spectroscopy is extremely useful when MR imaging fails to resolve certain clinical questions. Differentiating neoplasms from infectious processes and postradiation effects is often difficult, even with the most advanced MR technologies. In contrast, MR spectroscopy offers a noninvasive means of assessing the biochemical and metabolic processes in intracranial tissues without the ionizing radiation associated with both positron emission tomography and single-photon emission computed tomography imaging. The use of MR spectroscopy to evaluate patients with temporal lobe epilepsy is less well established but may be its most promising future application given the prevalence of the condition. With extensive ongoing investigations regarding the utility of the technique in a wide variety of neurologic, psychiatric, and head and neck disorders, MR spectroscopy will likely become an increasingly important component of MR imaging.
Key Words: epilepsy, infection, MR imaging, MR spectroscopy, neoplasms, radiation necrosis
Most neuroradiologic imaging techniques visualize both normal and abnormal anatomic structures to elucidate physiologic changes within a patient. Positron emission tomography (PET) and single-photon emission computed tomography (SPECT) have been used in a limited number of clinical situations and provide additional information about physiologic changes that routine cross-sectional imaging techniques cannot detect. Magnetic resonance (MR) spectroscopy provides similar information about biochemical and metabolic changes in disease processes but without the ionizing radiation of PET and SPECT. This article highlights some of the basic concepts underlying MR spectroscopy and several of its most common clinical applications.
Basic Principles of MR Spectroscopy
Different MR techniques use similar principles to obtain information from a tissue sample. Although information can be obtained from any nuclei with an odd atomic number, 1H is most commonly used in medical applications because it is far more prevalent within the human body than other nuclei with magnetic moments such as 31P and 13C. MR imaging techniques use gradient magnetic fields to provide spatial information to generate an image within a slice of tissue. In contrast, MR spectroscopy uses these fields to localize a specific volume of tissue for spectral analysis. Mathematically, the free induction decay signal from this volume is transformed into specific components of different amplitudes and frequencies. Each frequency corresponds to nuclei in a distinct magnetic environment, with the amplitude related to the number of nuclei within the volume in that particular state.
Two different techniques are commonly used to localize the desired volume for analysis: STEAM (stimulated echo acquisition mode) and PRESS (point-resolved spectroscopy).[22] Typically, the STEAM pulse sequence uses relatively short echo times (20 to 30 ms) and permits metabolites with short T2 relaxation times, such as myoinositol, glutamine/glutamate, and mobile lipids, to be examined.[27] For longer echo times (> 135 ms), PRESS is the volume-localization method of choice. Compared to STEAM, PRESS provides a two-fold gain in signal intensity and is less sensitive to patient motion.[5] Primarily due to its advantageous signal-to-noise ratio (SNR), PRESS is the volume localization method most often used in clinical spectroscopy. Both techniques require that the magnetic field over the analyzed volume be as homogeneous as possible. Inhomogeneity of the magnetic field broadens the resonance peaks, often making it impossible to distinguish among smaller adjacent peaks.
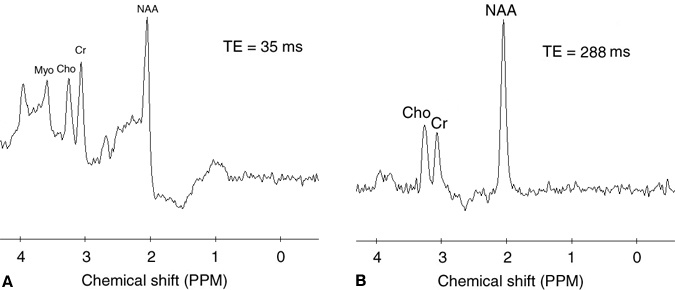
The spectra typically displayed are not expressed in absolute units of frequency (Hz) but rather in dimensionless units of parts per million (ppm). Spectra from different MR units can then be compared because the resonance frequencies are directly proportional to the strength of the magnetic field. Commonly encountered resonances in normal brain tissue include N-acetyl aspartate (NAA), choline, creatine, myoinositol, and glutamine/glutamate (Fig. 1). Lactic acid, lipids, acetate, succinate, and amino acids are other resonances often found in a variety of disease states.
NAA (2.0 ppm) is the largest peak in normal spectra. It is considered a marker of normal nerve cell body and axonal function. A decrease in its peak indicates axonal injury or neuronal loss.[27] Choline is a component of phospholipid metabolism. Increases in the choline peak (3.2 ppm) likely reflect an increase in membrane synthesis, an increase in the number of cells, or both.[5] Creatine (3.03 ppm) likely serves as a reserve for energy reservoirs and remains relatively stable even when disease is encountered. Myoinositol (3.56 ppm) is a metabolite involved in hormone-sensitive neuroreception; its peak increases in Alzheimer’s disease.[25] Glutamate and glutamine resonate together between 2.1 and 2.5 ppm. Glutamate is an excitatory neuro transmitter, and glutamine is in volved in the regulation of neurotransmitter activities.[24,32]
The lactate peak (1.32 ppm) consists of two distinct resonant peaks (a doublet), which reflect magnetic field interactions between adjacent protons (referred to as J-coupling). Typically, the lactate peak indicates the presence of carbohydrate catabolism.[30] A peak at 1.32 ppm can be confirmed as lactate by altering the echo time. At echo times of 35 and 288 ms, the lactate doublet is above baseline while it inverts below baseline when the echo time is 144 ms. Membrane lipids in the brain are seldom encountered unless echo times are short. When present, lipid resonances occur at 0.8, 1.2, 1.5, and 6.0 ppm. Acetate (1.9 ppm), succinate (2.4 ppm), and various amino acids (0.9 ppm) are bacterial metabolites that can be encountered in abscess cavities.
Clinical Applications of MR Spectroscopy
Radiation Necrosis Versus Recurrent Tumor
Radiation therapy is used to treat many tumors and often damages the vascular endothelium, causing tissue ischemia and necrosis. This effect most often follows the treatment of high-grade astrocytomas. On routine MR imaging studies, areas of radiation necrosis are characterized by irregular contrast enhancement around the margins of the surgical bed, often associated with edema, mass effect, and areas of cavitation. Unfortunately, the appearance of radiation necrosis is often indistinguishable from recurrent tumor. Distinguishing the two entities is important because their treatment strategies can differ. Radiation damage to brain parenchyma can be treated conservatively or with surgical resection while patients with recurrent tumor may also benefit from radiation therapy or chemotherapy.
Early work with PET, with reports of its almost perfect sensitivity and specificity, was encouraging. Ricci et al.,[28] however, retrospectively evaluated 84 patients and correlated PET findings with pathologic analysis in 31 patients. The sensitivity and specificity of PET for differentiating radiation necrosis from recurrent tumor ranged from 73 to 86% and 22 to 56%, respectively, depending on the choice of reference standard. The results with thallium-201 SPECT appear to be only slightly better. For distinguishing recurrent tumor from radiation necrosis after standard radiotherapy or gamma knife radiosurgery, its sensitivity ranged from 92 to 95% and its specificity from 60 to 67%.[21]
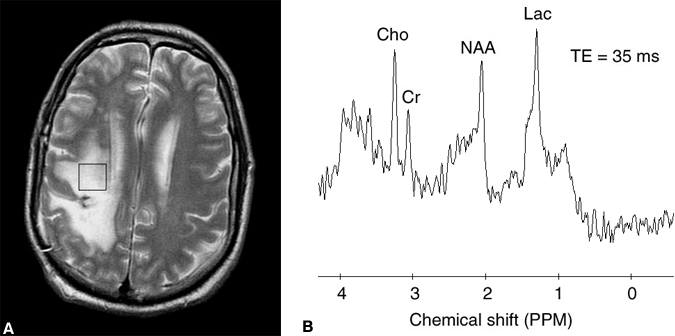
Initial reports of the ability of MR spectroscopy to discriminate recurrent tumor from postradiation effects have been encouraging. Ende et al.[14] used serial MR spectroscopy to examine 25 patients who had been treated at their institution for cerebral astrocytomas. Nine patients with a tumor recurrence had elevated ratios of choline/NAA and choline/creatine, and lactate was present (Fig. 2). Eight patients with radiation necrosis showed a marked decrease in their levels of NAA, choline, and creatine and had a broad intense peak between 0 and 2.0 ppm. This peak likely was produced by a combination of free fatty acids, lactate, and amino acids, all compounds associated with tissue necrosis. Wald et al.[33] reported a reduced level of choline in 12 patients with a glioblastoma multiforme after their treatment with brachytherapy. In four patients with clinical evidence of tumor progression, choline increased in areas that had previously appeared normal or necrotic. Prospective spectroscopy has also correctly identified five of seven residual or recurrent tumors and four of five cases of radiation necrosis.[31]
Ongoing large-scale studies comparing the different modalities of PET, SPECT, and MR spectroscopy should clarify some of the conflicting findings from prior studies. The wide availability of MR scanners to perform MR spectroscopy makes this modality more attractive than PET or SPECT for distinguishing radiation necrosis from recurrent tumor. Analysis of cerebral blood flow with MR perfusion imaging may also prove to be a useful technique.
Lymphoma Versus Toxoplasmosis in Acquired Immunodeficiency Syndrome
Neurologic abnormalities are common in patients with acquired immunodeficiency syndrome (AIDS). Routine MR imaging can reveal both focal and diffuse cerebral abnormalities. Classic examples of toxoplasmosis and cerebral lymphoma are easy to distinguish. Toxoplasmosis is typically associated with multiple ring-enhancing lesions; lymphoma classically appears as a single enhancing lesion in a periventricular location. However, the appearance of the two entities often overlaps significantly. There may be only one toxoplasmic lesion, and lymphoma can be multifocal and associated with central necrosis, especially in immunocompromised patients.
Early, accurate, noninvasive identification of these two processes is important for many reasons. Typically, AIDS patients with ring-enhancing lesions on MR imaging are treated empirically for toxoplasmosis. The brain is biopsied if patients show no clinical or radiographic response to medical therapy within 2 weeks. Antitoxoplasmic therapy, however, is not benign. Most patients experience allergic reactions or hematologic toxicity.[8] Furthermore, some patients with toxoplasmosis may not improve within 2 weeks. In addition, patients with lymphoma have a very brief life expectancy after their initial presentation. If patients with fulminant tumors deteriorate rapidly, brain biopsy may no longer be an option.
Several noninvasive modalities have been used to distinguish toxoplasmosis from lymphoma in AIDS patients, but the results have been variable. On PET, glucose uptake increases in lymphomas and decreases in toxoplasmosis, but this technique is expensive and not widely available.[17] With SPECT the uptake of thallium-201 increases in tumors and decreases in infections, but its sensitivity and specificity are limited. Although conflicting results have been reported, several investigators have shown that MR spectroscopy reliably differentiates toxoplasmosis from lymphoma in AIDS patients.[9,10]
Chang et al.[9] performed MR spectroscopy on 35 AIDS-related lesions in 26 patients, including 11 toxoplasmic abscesses and eight lymphomas. By assessing the normalized peak areas of NAA, creatine, choline, myoinositol, and lactate, the correct diagnosis was predicted with an accuracy of 84 to 94%. Toxoplasmosis lesions were associated with a marked increase in the resonance of lactate and the absence of normal brain metabolites. Lymphomas were associated with moderate increases in lactate and lipids, moderate decreases in NAA and creatine, and a marked increase in choline. The increase in the choline peak likely reflects the rapid synthesis and turnover of all membranes in the highly cellular, rapidly growing, lymphomatous tumors.
Chinn et al.[10] studied 27 lesions (18 toxoplasmic abscesses and 9 lymphomas), but their results were less encouraging. They concluded that their spectra were unable to differentiate the two processes reliably using both visual and quantitative assessments. This study, however, used different scanning parameters, and the investigators acknowledged that the spectra acquired from the lesions were technically poor, possibly related to patient motion. Although further investigation is needed, MR spectroscopy may be a valuable tool for distinguishing toxoplasmosis from lymphoma in AIDS patients and may help direct clinicians toward proper therapy earlier than previously possible.
Abscess Versus Cystic or Necrotic Tumor
Although the mortality and morbidity rates associated with a pyogenic brain abscess have decreased significantly since the advent of cross-sectional imaging, differentiating an abscess from tumors such as high-grade gliomas and metastases with routine MR imaging techniques can still be challenging. Shared features such as peripheral rim enhancement, central cystic components, and adjacent edema often preclude an accurate preoperative diagnosis. Early identification of small abscesses is potentially useful because those less than 2.0 cm in diameter may be treated by medical management alone.[26] Recent studies have demonstrated the promise of diffusion-weighted imaging, but MR spectroscopy has already proven reliable for distinguishing the chemical compositions of abscess and tumor.
Differentiation of the contents of abscess cavities from those of necrotic or cystic tumors is based on the presence of multiple bacterial metabolites (lactate, acetate, succinate, amino acids) within the abscess. A wide variety of amino acids and other compounds have been detected within abscess cavities, likely reflecting differences in both the spectroscopic parameters used to obtain the chemical spectra and differences in the causative organisms.
Martinez-Perez et al.[23] performed MR spectroscopy in two patients with lesions subsequently documented to be abscesses by surgical biopsy. The abscesses (both caused by Streptococcus species) showed resonances attributable to acetate, lactate, multiple fatty acids, and amino acids (alanine, glycine, valine, leucine, isoleucine, and phenylalanine). Subsequent in vitro spectroscopy of the aspirated abscess contents yielded similar findings, with additional minor resonances attributed to succinate and other amino acids. Acetate and phenylalanine were proposed as potential specific markers of abscesses.[23]
Kim et al.[20] reported the results of MR spectroscopy in 14 patients with ring-shaped areas of contrast enhancement indicative of cystic or necrotic masses. Seven patients had pyogenic brain abscesses and seven patients had cystic or necrotic tumors (five had a glioblastoma and one each had a pilocytic astrocytoma and metastatic lung cancer). Six abscesses demonstrated resonances attributed to lactate, acetate, succinate, amino acids (alanine, valine, leucine), and several unidentified metabolites. In six of the cystic or necrotic tumors, the only resonance detected was attributed to lactate. A subsequent study by the same group[7] analyzing eight abscesses with various other intracranial cystic masses found resonances from lactate, acetate, succinate, and amino acids within the abscesses. The same spectral pattern was also observed in four cysts caused by cysticercosis.
Dev et al.[13] used MR spectroscopy to evaluate 24 patients with pyogenic brain abscesses, 22 of whom were treated with combined medical and surgical therapy and 2 of whom were treated with medical therapy alone. High-resolution MR spectroscopy was performed on 15 abscess samples to confirm the identity of the resonances obtained in vivo. Fourteen patients (12 treated with combined therapy, 2 treated medically) underwent post aspiration in vivo studies. Lactate and amino acids (alanine, valine, leucine, isoleucine) were found in all abscesses, regardless of the timing of spectroscopy after the onset of combined medical and surgical therapy. Other resonances detected were acetate, pyruvate, lipids, glycine, and glutamate/glutamine. No succinate was detected. In abscesses with pyruvate and acetate resonances, these compounds were no longer detected after 1 week of combined treatment.
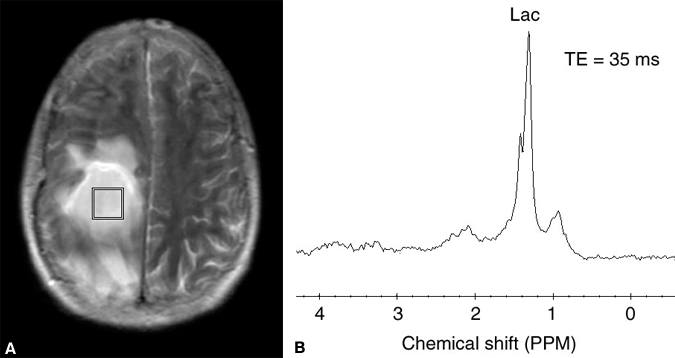
Burtscher and Holtas[4] examined three patients with brain abscess, both before and at different times after the institution of antibiotic therapy. In two patients, the first examined before and the second one day after initiation of antibiotics, the spectra were similar to prior studies: Resonances were attributed to acetate, lactate, pyruvate, succinate, and amino acids (valine, leucine, isoleucine). In two patients, spectroscopy performed after several weeks of antibiotic therapy demonstrated only a single resonance, which was attributed to lactate. This finding suggests that spectroscopy could be used to evaluate changes after the institution of therapy (Fig. 3).
Grand et al.[16] used two-dimensional MR spectroscopy and liquid chromatography to determine amino acid concentrations in 13 abscesses within the brain and at other locations. The concentrations were compared to the results of in vivo spectroscopy performed in 34 patients with cystic intracranial lesions. The most commonly detected resonances in abscesses were lactate, acetate, succinate, and amino acids (alanine, valine, leucine, isoleucine). Acetate and succinate were suggested as possible specific markers for abscess. Interestingly, amino acids were detected in minute concentrations in several samples of cystic or necrotic tumors although they were not detected using in vivo spectroscopy. This outcome likely reflects the much lower signal-to-noise ratio associated with the in vivo technique.
The results of these studies demonstrate that MR spectroscopy may be a useful noninvasive diagnostic tool for the evaluation of cystic intracranial lesions. Acetate and succinate resonances appear to be specific markers for infection and can be present in pyogenic abscess cavities, cysticercosis, and toxoplasmosis.[6] Amino acids have been detected in low concentrations in cystic or necrotic tumors, but their presence is also highly suggestive of abscess. Although antibiotic therapy has been associated with spectral conversion to a single lactate resonance, additional studies are needed to confirm and determine the usefulness of this finding. Future studies also may lead to the identification of metabolites associated with specific microorganisms; succinate has already been suggested as a marker of anaerobic infection.[15]
Localization of Seizure Foci in Temporal Lobe Epilepsy
Seizures are a nonspecific symptom of underlying neurologic abnormality. They occur not only in patients with focal cerebral lesions but also in those with fever, an acute central nervous system infection, hypoglycemia, and other conditions. A percentage of patients with seizures experience multiple spontaneous episodes without an identifiable cause and are classified as having epilepsy. Epilepsy is a common condition in the Western world, with an estimated prevalence of 0.5 to 1.0%.[1] Approximately a fourth of these patients become refractory to medical therapy and develop major complications and disability if left untreated.[34]
Classification of seizure types is based on the clinical description of the seizure events and the pattern of electroencephalographic (EEG) abnormalities both during (ictal) and between (interictal) seizures. The most commonly accepted classification scheme, based on the International Classification,[11] divides epilepsy into generalized seizures (those affecting the entire body) and partial seizures (those affecting only a portion of the body). Partial seizures can be further subclassified into simple (without impairment of consciousness) and complex (with impairment of consciousness).
Temporal lobe epilepsy is the dominant form of complex partial epilepsy. It is often associated with hippo campal sclerosis, which is characterized by gliosis and atrophic change in the affected hippocampus. MR imaging using standard protocols can detect other causes of seizures such as tumors, vascular malformations, and migrational abnormalities. Thin coronal slices through the hippocampal formations, however, are needed to detect the characteristic features of hippocampal sclerosis, which are decreased hippocampal volume and abnormal signal intensity. Surgical treatment with a modified amygdalohippocampectomy usually eliminates seizures in these patients although patients occasionally suffer devastating verbal memory deficits as a consequence of the surgery.
Proper patient selection is critical in maximizing the success of surgical therapy while minimizing associated morbidity. A thorough presurgical evaluation is usually performed, with routine clinical evaluation, MR imaging, surface EEG, and neuropsychological testing. Ideally, all of the in formation is consistent with an epi leptogenic focus in one of the temporal lobes. In as many as 20% of cases, however, the clinical and surface EEG features of temporal lobe epilepsy falsely lateralize the hemisphere of seizure onset.[29] Occasionally, MR imaging demonstrates bilateral abnormalities or no abnormalities at all. Without additional noninvasive imaging techniques such as PET and MR spectroscopy, patients would be subjected to the risks of placing invasive depth electrodes. Noninvasive methods of lateralizing the seizure focus are desirable to minimize such complications and to reduce the possibility of postoperative memory deficits.
The use of MR spectroscopy in the evaluation of temporal lobe epilepsy has been associated with encouraging results. In 1993 Hugg et al.[19] first showed that MR spectroscopy could be used to lateralize seizure foci in patients with temporal lobe epilepsy. The NAA/(choline + creatine) ratio was decreased 20% in the temporal lobe ipsilateral to the seizure focus in all patients. Connelly et al.[12] evaluated the medial temporal lobes of 25 patients with temporal lobe epilepsy and compared their spectra to normal controls. The temporal lobes ipsilateral to the seizure focus showed a mean reduction of 22% in the NAA peak with a 25% increase in choline and a 15% increase in creatine. The contralateral temporal lobes demonstrated similar effects of lesser magnitude.
ratio (0.57) confirms sclerosis of the right hippocampus.“]
Achten et al.[2] examined 21 patients with temporal lobe epilepsy and 12 healthy subjects to investigate which absolute metabolite concentration or ratio of metabolites would best localize a seizure focus in the former group. The normalized NAA concentration [NAA] decreased a mean of 31% ipsilateral to the seizure focus and 15% on the contralateral side. The most consistent parameter for correct localization was the NAA/(choline + creatine) ratio, followed by [NAA]. The bilateral presence of multiple abnormal values led the authors to introduce an adequate asymmetry index, which improved correct lateralization in patients with bilateral abnormal values. Of the 21 patients, 17 were lateralized concordantly with EEG and MR findings using the NAA/(choline + creatine) ratio, which was considered pathologic if the value was less than 0.71 (Fig. 4).
PET has been used extensively in epilepsy research in attempts to localize regions of altered cell metabolism. The incidence of hypometabolism in the temporal lobe ipsilateral to the seizure focus can range from 60 to 90%.[1] Another study by Achten et al.[3] compared the metabolic information obtained from PET studies to MR spectroscopy in 29 patients with temporal lobe epilepsy. In 25 patients whose seizure onset could be localized to one temporal lobe, 24 (96%) showed ipsilateral localization with either technique. However, concordant lateralization with both techniques was only possible in 14 patients (56%). All patients with hypometabolic temporal lobes on PET also had decreased NAA/(choline + creatine) ratios. Still, seizures were correctly lateralized with MR spectroscopy in five patients who had negative PET studies. Although more investigations are needed, MR spectroscopy may be sensitive enough to lateralize seizure foci in patients with temporal lobe epilepsy, and it may be the best initial study to achieve concordance of clinical, imaging, EEG, and neuropsychologic information.
Conclusions
Because of the large number of patients with temporal lobe epilepsy and the small number of patients studied, more work is needed to establish the role of MR spectroscopy in guiding clinical decision-making and surgical planning. In particular, the significance of bilateral temporal lobe abnormalities must be investigated further. Chemical shift imaging, which allows detection of multiple spectra within a large voxel, is equivalent to single-voxel MR spectroscopy in terms of detecting hippocampal abnormalities. In the future, it may be the favored spectroscopic technique.[18] Because MR units are widely available and the prevalence of temporal lobe epilepsy is high, the most common future application of MR spectroscopy may be the evaluation of such patients.
References
- Achten E: Aspects of proton MR spectroscopy in the seizure patient. Neuroimaging Clin N Am 8:849-862, 1998
- Achten E, Boon P, Van De Kerckhove T, et al: Value of single-voxel proton MR spectroscopy in temporal lobe epilepsy. AJNR Am J Neuroradiol 18:1131-1139, 1997
- Achten E, Santens P, Boon P, et al: Single-voxel proton MR spectroscopy and positron emission tomography for lateralization of refractory temporal lobe epilepsy. AJNR Am J Neuroradiol 19:1-8, 1998
- Burtscher IM, Holtas S: In vivo proton MR spectroscopy of untreated and treated brain abscesses. AJNR Am J Neuroradiol 20:1049-1953, 1999
- Castillo M, Kwock L, Mukherji SK: Clinical applications of proton MR spectroscopy. AJNR Am J Neuroradiol 17:1-15, 1996
- Castillo M, Kwock L, Scatliff J, et al: Proton MR spectroscopy in neoplastic and non-neoplastic brain disorders. Magn Reson Imaging Clin N Am 6:1-20, 1998
- Chang KH, Song IC, Kim SH, et al: In vivo single-voxel proton MR spectroscopy in intracranial cystic masses. AJNR Am J Neuroradiol 19:401-405, 1998
- Chang L, McBride D, Miller BL, et al: Localized in vivo 1H magnetic resonance spectroscopy and in vitro analyses of heterogeneous brain tumors. J Neuroimaging 5:157-163, 1995
- Chang L, Miller BL, McBride D, et al: Brain lesions in patients with AIDS: H-1 MR spectroscopy. Radiology 197:525-531, 1995
- Chinn RJ, Wilkinson ID, Hall-Craggs MA, et al: Toxo plasmosis and primary central nervous system lymphoma in HIV infection: Diagnosis with MR spectroscopy. Radiology 197:649-654, 1995
- Commission on Classification and Terminology of the International League Against Epilepsy: Proposal for revised clinical and electroencephalographic classification of epileptic seizures. Epilepsia 22:489-501, 1981
- Connelly A, Van Paesschen W, Porter DA, et al: Proton magnetic resonance spectroscopy in MRI-negative temporal lobe epilepsy. Neurology 51:61-66, 1998
- Dev R, Gupta RK, Poptani H, et al: Role of in vivo proton magnetic resonance spectroscopy in the diagnosis and management of brain abscesses. Neurosurgery 42:37-43, 1998
- Ende J, Scatliff JH, Powers S, et al: Spectral proton and P-31 MR spectroscopy patterns of treated human brain tumors. Annual Meeting of the Society of Magnetic Resonance in Medicine, 1992
- Gorbach SL, Mayhew JW, Bartlett JG, et al: Rapid diagnosis of anaerobic infections by direct gas-liquid chromatography of clinical specimens. J Clin Invest 57:478-484, 1976
- Grand S, Lai ES, Esteve F, et al: In vivo 1H MRS of brain abscesses versus necrotic brain tumors. Neurology 47:846-848, 1996
- Hoffman JM, Waskin HA, Schifter T, et al: FDG-PET in differentiating lymphoma from nonmalignant central nervous system lesions in patients with AIDS. J Nucl Med 34:567-575, 1993
- Hsu YY, Chang C, Chang CN, et al: Proton MR spectroscopy in patients with complex partial seizures: Single-voxel spectroscopy versus chemical-shift imaging. AJNR Am J Neuroradiol 20:643-651, 1999
- Hugg JW, Laxer KD, Matson GB, et al: Neuron loss localizes human temporal lobe epilepsy by in vivo proton magnetic resonance spectroscopic imaging. Ann Neurol 34:788-794, 1993
- Kim SH, Chang KH, Song IC, et al: Brain abscess and brain tumor: Discrimination with in vivo H-1 MR spectroscopy. Radiology 204:239-245, 1997
- Kline JL, Noto RB, Glantz M: Single-photon emission CT in the evaluation of recurrent brain tumor in patients treated with gamma knife radiosurgery or conventional radiation therapy. AJNR Am J Neuroradiol 17:1681-1686, 1996
- Kwock L: Localized MR spectroscopy: Basic principles. Neuroimaging Clin N Am 8:713-731, 1998
- Martinez-Perez I, Moreno A, Alonso J, et al: Diagnosis of brain abscess by magnetic resonance spectroscopy. Report of two cases. J Neurosurg 86:708-713, 1997
- Michaelis T, Merboldt KD, Bruhn H, et al: Absolute concentrations of metabolites in the adult human brain in vivo: Quantification of localized proton MR spectra. Radiology 187:219-227, 1993
- Miller BL, Moats RA, Shonk T, et al: Alzheimer disease: Depiction of increased cerebral myo-inositol with proton MR spectroscopy. Radiology 187:433-437, 1993
- Osenbach RK, Loftus CM: Diagnosis and management of brain abscess. Neurosurg Clin N Am 3:403-420, 1992
- Rand SD, Prost R, Li SJ: Proton MR spectroscopy of the brain. Neuroimaging Clin N Am 9:379-395, 1999
- Ricci PE, Karis JP, Heiserman JE, et al: Differentiating recurrent tumor from radiation necrosis: Time for re-evaluation of positron emission tomography? AJNR Am J Neuroradiol 19:407-413, 1998
- Risinger MW, Engel J, Jr., Van Ness PC, et al: Ictal localization of temporal lobe seizures with scalp/sphenoidal recordings. Neurology 39:1288-1293, 1989
- Sanders JA: Magnetic resonance spectroscopy, in Orrison WW, Lewine JD, Sanders JA, et al (eds): Functional Brain Imaging. St. Louis: Mosby, 1995, pp 419-467
- Taylor JS, Langston JW, Reddick WE, et al: Clinical value of proton magnetic resonance spectroscopy for differentiating recurrent or residual brain tumor from delayed cerebral necrosis. Int J Radiat Oncol Biol Phys 36:1251-1261, 1996
- van der Knaap MS, Ross B, Valk J: Uses of MR in inborn errors of metabolism, in Kucharczyk J, Mosely M, Barkovich AJ (eds): Magnetic Resonance Neuroimaging. Boca Raton: CRC Press, 1994, pp 245-318
- Wald LL, Nelson SJ, Day MR, et al: Serial proton magnetic resonance spectroscopy imaging of glioblastoma multiforme after brachytherapy. J Neurosurg 87:525-534, 1997
- Ware AA, Jr.: Perspectives of surgical therapy of epilepsy, in Ward AA, Penry JK, Purura D (eds): Epilepsy. New York: Raven, 1983